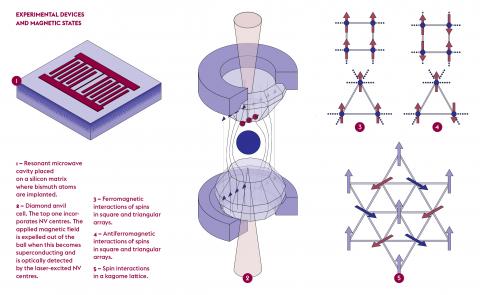
Different states of condensed matter
(This article was originally published in L'Edition No.14)
Physicists of condensed matter at Université Paris-Saclay set out to conquer new electronic and magnetic properties of matter.
“Oh! endless collage, shifting planes, the bleeding sun, the deep sea scattered with sloping sails. Matter upon matter...you could dissolve in it,” exclaimed painter Paul Klee. This description of the artist’s pictorial quest also evokes a beautiful and lyrical metaphor for current research into condensed matter physics – a field of research that explores matter in a state where atoms are spatially correlated.
Some materials – both natural or artificial – and behaviours discovered in recent years have such original and unexpected microscopic and macroscopic properties that physicists are once again stirred into action. Properties such as superconductivity, ferromagnetism and antiferromagnetism have challenged many accepted representations, and researchers at Université Paris-Saclay are striving to understand them. “Oh perfect kagome lattice of spins, frustrated geometry, microwave photon cooling, diamond-encased magnetometers. Magnetic field and pressure on matter; until it melts.” would be an apt declaration by specialists.
Magnetic behaviour under pressure
On a scale smaller than the atom, matter consists of atomic nuclei (composed of protons and neutrons) and electrons which participate in a concert of interactions. They are associated with various characteristics, such as mass or electrical charge. But it is their spin, a kind of internal mini-magnet, and its interactions with neighbouring counterparts, that are responsible for some of the most remarkable properties of matter. When placed in an external magnetic field, the electronic spins of some metallic materials – such as iron, nickel, or cobalt – are parallel. They develop strong magnetic properties, called ferromagnetism.
Jean-François Roch from the Light, Material and Interfaces Laboratory (LUMIN – UPSaclay, ENS Paris-Saclay, CNRS) and his colleague Thierry Debuisschert from Thales R&T investigated the magnetic behaviour of iron under high pressure. “The metal loses its magnetism between 15 and 20 GPa. The crystalline phase changes and the magnetic order that determines spin organisation disappears,” explains the researcher. Working with Paul Loubeyre, affiliate professor at ENS Paris- Saclay and researcher at CEA-DAM, his team has developed a new, more sensitive method for detecting the phenomenon, making it possible to visualise magnetic properties using optical and spectroscopic measurements, and crystallographic ones by X-ray diffraction measurements.
The method uses a press with two diamond anvils sharpened into tips. On one of the tips perfectly controlled flaws, called nitrogenvacancy (NV) centres, are distributed. Inserted into the diamond using a focused ion beam microscope, these NV centres act as atomic size magnetometers, capable of measuring magnetic resonance of sample spins.
“In the experiment, a magnetic field attracts an iron ball placed between the two anvils. As pressure is increased, the magnetic transition of the iron is observed: the higher the pressure, the more the magnetic field detected by the NV centres decreases, until it disappears,” says Jean-François Roch. “When pressure is lowered, magnetisation returns, displaying a hysteresis. The delay is due to the gradual switching of the magnetic domains in the iron ball.”
New metal superconductors
One of the major challenges in research into materials under high pressure is the emergence of superconductivity. This is due to the loss of electrical resistivity of materials, which begin to expel an applied magnetic field. This is called the Meissner effect. Thanks to their technique, Jean-François Roch and his team have detected this characteristic distortion of field lines when in the vicinity of a superconductor. “We used magnesium bromide (MgB2), a known superconductor, and tracked its superconductivity transition: at 7 GPa of pressure, superconductivity disappeared when the temperature exceeded 30 K.”
With supposedly quantum properties, metallic hydrogen is the Holy Grail for this type of research: the simplest element in the universe, it is supposed to become superconductive during metallic transition. This phenomena can be observed by applying such high pressure that the diamond anvils invariably break at 400 GPa, just before the hydrogen becomes metallic. Recently, Paul Loubeyre and his team at CEA-DAM reached pressures of 600 GPa with diamond anvils with machined torus tips. Using the SMIS beamline of the SOLEIL synchrotron, they observed metallic hydrogen formed at 425 GPa of pressure. This was unprecedented. Jean-François Roch and Paul Loubeyre are now turning their focus to metal hydrides, with properties similar to metallic hydrogen but metastable: these hydrogen-rich materials exhibit superconductive behaviour at near-ambient temperature under lower pressure. “Exploring and studying new compositions will require torus-shaped diamond anvils with deeper NV centres,” acknowledges Jean-François Roch.
Dancing liquid spins
The spins of ferromagnetic materials become parallel when an external magnetic field is applied to them, but antiferromagnetic materials do not present the same pattern under the same conditions. Their spins only align under a critical temperature, but they do it antiparallel, in alternate directions. The overall magnetisation of the material remains zero.
Atypical materials with certain antiferromagnetic characteristics are the focus of research by Philippe Mendels and Fabrice Bert of the Laboratory of Solid Physics (LPS – UPSaclay, CNRS). They are known as quantum spin liquids, but are not exactly liquid. They are named after their particular magnetic state: they are in a state of dynamic disorder, even at very low temperatures close to absolute zero (0 K, -273.15 °C) where the system should be ordered or frozen. This is due to the strange phenomenon called geometrical frustration. Due to the arrangement of atoms in a kagome lattice – a triangular network of Stars of David – electronic spins fail to achieve the required antiparallel alignment, and some of their interactions remain frustrated.
Subject of a purely theoretical description until recently, spin liquids now have a prize paragon: herbertsmithite (ZnCu3(OH)6Cl2). This mineral, discovered in 1972, has original properties that were confirmed in 2012, by the LPS among others. “Synthesised, this mineral demonstrates the same properties as quantum spin liquids. We observed the absence of magnetic order and magnetisation at very low temperatures, to 20 mK, whereas spins are typically ordered at 200 K,” explains Philippe Mendels. The copper atoms (Cu) in this compound organise themselves in a kagome lattice.
In the heart of atoms
To penetrate atoms and explore their magnetic phenomena, researchers use nuclear magnetic resonance spectroscopy (NMR). The technique depends on the ability of certain atomic nuclei to interact with a magnetic field. These are those with a nuclear spin – they present an odd number of protons or neutrons, or both – like the Oxygen-17 isotope (17O). Initially, the nuclear spins are aligned with an external magnetic field and then stimulated with a pulsed radiofrequency. The emission of electromagnetic waves when equilibrium is returned constitutes the detected signal.
In the case of herbertsmithite, researchers have recently used this technique to determine the energy required by the system to transform from ground state to excited state. “We showed there is no rise in energy. A minute temperature just above absolute zero is sufficient to excite the system. The system then becomes slightly magnetic, which increases with temperature,” explains Philippe Mendels.
Using experience
The challenge for researchers is to develop this material, to understand it better, and to exploit its amazing properties, with a view to generating new physical concepts. “What are the effects of possible changes to the kagome lattice on the properties of the material?” wonders Philippe Mendels. “For example, by replacing certain atoms, we will be able to observe if the kagome lattice is preserved, and distinguish properties caused by copper atoms from those due to other atoms.” Fabrice Bert adds: “Herbertsmithite is a very good insulator. If we succeed in adding free moving charges, we may discover very original transport properties.”
One thing is for sure: “The discovery of herbertsmithite was fundamental. It has allowed us to test theoretical predictions established in the 80s about spin liquids in experiments,” says Philippe Mendels. It is now a very fast-moving field. Other materials have joined this strange family, such as Sr-vesignieite or DQVOF, a vanadium oxyfluoride that does not exist in nature. “It is often the discovery of new materials that triggers our experiments,” agrees Philippe Mendels.
When spins cool
In the Condensed Matter Physics laboratory (SPEC – UPSaclay, CEA, CNRS) in the Quantronics group, other spins with strange behaviour in a crystal are attracting the attention of Patrice Bertet and his colleagues. Their recent work promises to boost the detection of signals by NMR or by electronic paramagnetic resonance (EPR), the equivalent of NMR for electronic spins. “It is the polarisation (or alignment) of spins, initiated by the application of an external magnetic field, when performing a magnetic resonance experiment, that determines the strength of the signal transmitted during their relaxation after excitation. The signal coincides with the temperature of the crystal in which the spins are located: it is polarisation at thermal equilibrium,” explains Patrice Bertet. “To increase the signal, we try to increase either the intensity of the applied magnetic field, or the polarisation of spins beyond the authorised limited temperature, which is called hyperpolarisation.” Following this last principle, researchers from SPEC developed an original radiative cooling method and succeeded in hyperpolarising electronic spins at a temperature lower than that of the sample.
To do so, they reversed the balance between the mechanisms used by the spins to recover thermal equilibrium. “When a system is excited in condensed matter physics – such as nuclear or electronic spins in an NMR or EPR experiment – balance can be achieved by exchanging a quantum of energy with the environment: either the emission of a photon (radiation) or a phonon (vibration),” says Patrice Bertet. Spontaneous emissions of photons are possible, but very rare: “At low temperatures, an electronic spin spontaneously emits a photon every 1012 seconds – that’s every 32,000 years!” The main relaxation mechanism used by spins is coupling with phonons, which thermalise at the temperature of the crystal structure. “It is the temperature required to make atoms vibrate.” To decrease even further, relaxation by spontaneous emission of photons must increase.
Using microwave containment
The researchers succeeded using a resonant microwave cavity. They first inserted bismuth atoms into a silicon crystal matrix, then coupled the electronic spins supplied by the system to a concentrated microwave electromagnetic field in the resonator. Using a coaxial cable, they connected the resonator initially at a temperature of 850 mK to a 20 mK cooled resistor. “Using the microwave resonator, we increased interaction between spins and the electromagnetic field until microwave photon emission became the dominant mechanism of relaxation toward the equilibrium of spins. This is called the Purcell effect: spins thermically decouple from the crystal. Their temperature is entirely determined by the microwave field, not by the phonons.” By measuring the RPE signal – 2.3 times higher - the researchers concluded that the microwave radiation cooled the spins to a temperature of 350 mK.
The method does not apply directly to nuclear spins, but it is possible to combine it with the dynamic nuclear polarization (DNP) technique, which uses electronic spins to hyperpolarise nuclear spins. To go beyond the physical limit imposed by the temperature of the cold source, researchers plan to use a mechanical or electrical oscillator. “It will be possible to actively cool spins to a temperature much lower than the cryostat,” says Patrice Bertet. The future of the search for new properties is assured.
Publications
- Albanese B. et al., Radiative cooling of a spin ensemble. Nature Physics. 16, 751-755 (2020).
- Khuntia, P. et al., Gapless ground state in the archetypal quantum kagome antiferromagnet ZnCu3(OH)6Cl2. Nature Physics. 16, 469-474 (2020).
- Lesik M. et al., Magnetic measurements on micrometer-sized samples under high pressure using designed NV centers. Science. 366, 6471, (2019).